2. A Basic Sketch of Endogenous, Self-Sustained Oscillators
At the heart of a circadian clock is an endogenous, self-sustained oscillator (ESSO). An oscillator is a mechanism that fluctuates between different states repeatedly and with a stable frequency. Two familiar examples are the swinging of a pendulum or pulsing of a beating heart. Because we are dealing with circadian clocks, the period or length of time that it takes the clock to oscillate is about 24 hours (again: circa-dian, about a day). An oscillator is self-sustained if it can continue to count off these ~1-day-long periods indefinitely without damping out. Unlike a pendulum clock which eventually runs down and needs to be started again, a self-sustained clock requires no external event for it to continue to oscillate. (A heart would be considered self-sustained since it persists throughout the life-span). An endogenous oscillator is one that is internal to the organism and not a reflection environmental inputs.
It is useful to compare and contrast a biological ESSO with artificial time-keeping devices. An hourglass or sand timer is a potentially very useful device for keeping track of time, but it is not an ESSO. When an hourglass is turned over, the duration it takes for the sand to run down may be used to measure a determinate length of time. However, an hourglass is not a self-sustained method of keeping time: once the sand has run down, the hourglass cannot, on its own, count down another duration of time again. It must be manually turned over and reset – it requires an outside influence to count down another length of time. Similarly, an hourglass is not an oscillator: the sand only runs in one direction, and then time-keeping ceases. In contrast, the mechanism that runs a circadian clock, as an ESSO, does not cease its time-keeping activity, and does not require an outside influence to continue keeping time.

A standard analog mechanical clock or wristwatch may be regarded as an oscillator. The big and small hands of the clock rotate or cycle around the clock-face throughout the day. For standard clock-devices, the cycle of the hour-hand has a period of 12 hours, not 24-hours: we distinguish AM and PM in order to count off a full 24-hour cycle using such clocks. It might have been very handy for early humans to have such an external or exogenous time-keeping device – and to find a way of reading it at night. But no such measuring device was readily available in the natural environment; luckily, our ancestors had evolved an endogenous clock.
Chronobiologists have developed a number of relatively standardized formats for presenting data in support of the function of a circadian clock. In the remainder of this section, we will introduce the reader to these formats, and discuss some pertinent examples.
2.1 Telling Time in Chronobiology
We are so used to telling time in conventional, human-centric ways that it can be difficult for us to speak clearly about how an organism keeps time using its own circadian clock. For example, consider again de Mairain’s or de Candolle’s experiments involving Mimosa plants. These plants normally exist in an environmental light-dark cycle: circadian biologists will often summarize this as an “LD” cycle (L for light and D for dark). But de Mairan put them into constant darkness (which circadian biologists shorten “DD”) and de Candolle put them into constant light (“LL”). When the plants were in constant light, their leaves opened and closed rhythmically with a periodicity of 22.5 hours. This is one important piece of information: the free-running period of the rhythm was not actually 24 hours. And so, “for the Mimosa,” “one day” is 22.5 hours, and not 24 hours (when there are no external cues to entrain it). One can easily see how confusion could arise if we did not have a standardized vocabulary for distinguishing the Mimosa’s endogenous day from an actual solar day.
Chronobiologists have developed such a vocabulary. Chronobiologists speak of “subjective time,” or, more frequently, circadian time (CT) whenever they are discussing the predicted length of a day from the viewpoint of an organism’s un-entrained, or free-running, circadian clock. For example, for the free-running Mimosas, one circadian day = 22.5 hours. This is not a full, actual day, but it is the length of time that the free-running Mimosa “thinks” is a day. Just as a solar day is divided into 24 equal portions that we call “hours,” a circadian day is divided into 24 equal portions that we designate as “circadian hours.”
CT is invoked only when an organism is free-running: if the organism is entrained to an environmental rhythm, then there will be no need to speak of CT, because the organism will be tracking an environmental time-cycle. But there is an additional complication. Just as de Mairan and de Candolle imposed an artificial manipulation to remove all external time-cues, putting their Mimosa plants in constant conditions in order to determine their free-running period, so likewise, chronobiologists often impose an artificial environmental time-cycle on an organism. Normally, an organism living in natural conditions has access to some external, environmental cues that indicate the present time of day. In the most straightforward case, this is the light-dark cycle of a day as the sun rises and sets. The rhythmic changes of light and dark report the current time to most organisms. The light and dark are, as one would say in German, an external zeitgeber (“time-giver”). Because influential figures in the history of chronobiology were German, (See Box 2) the field has retained this way of speaking. A zeitgeber is any environmental cue that can be used by an organism to align its endogenous rhythm with the external day-night cycle. So far we have focused mainly on light as it is the most common zeitgeber, but there are other zeitgebers as well. Because laboratory settings can simulate a wide range of conditions, the zeitgeber often does not indicate the actual day-night cycle of the environment outside.
Let us consider an example. Suppose that you run a laboratory that studies the circadian rhythms of mice. You are especially interested in what mice do when they are awake and active. Now you have a problem: you, as a human, are predominantly diurnal: your wakeful and active period occurs during the day, when the sun is up. In contrast, your mice are predominantly nocturnal: their wakeful and active period occurs during the night, when the sun is down. You could expose your mice to the local day-night cycle and simply decide that in order to gather your data, you have to stay up all night so as to observe the mice in their active phase. (You use a night-vision camera to see what the mice are up to in darkness). But humans suffer a number of impairments if they try to stay awake all night, because humans’ circadian clocks act to schedule their wakeful, active processes during the day (for a recent review, see Roenneberg & Merrow 2016). It would be healthier for you, and more convenient, if you played a trick on the mice. Instead of exposing them to the local day-night cycle, you could impose an artificial day-night cycle on them – after all, they live their entire lives in your laboratory. You decide to place the mice in a portion of your lab that is subjected to the following lighting scheme: when it is actually light outside during the day, the mice experience only darkness, and when it is actually dark outside during the night, the mice experience only light. The convenient consequence will be that when you, as a diurnal organism, are at the lab (during the day), the mice, as nocturnal organisms, will be exposed only to darkness (and so will be active, since they think it is night). And while you, as a diurnal organism, are at home at night the nocturnal mice will be sleeping in the artificial light that is telling their circadian clocks that it is day. If you really need to see your mice personally, data-collection would be considerably easier for you under this lighting scheme.
This is a clever bit of laboratory practice, and it will make your life much easier. But note what has happened: the LD cycle which the organism is exposed to is not the actual, local day-night cycle. You have exposed the mice to zeitgebers which are not indicative of actual environmental time: instead you have imposed an artificial environmental cycle upon the organism. As a result, it will not be possible to communicate what lighting condition the mice are experiencing if you simply cite one of your favorite human clock-devices. For example, it is irrelevant that you observed a mouse being active “at 4pm local time.” What is relevant is that you made an observation near the end of that mouse’s (artificially induced) nighttime phase. Chronobiologists often impose this kind of artificial LD cycle upon an organism. In order to communicate clearly, and in a relevant way, about when observations occurred, chronobiologists speak of zeitgeber time (ZT). It should never be assumed that ZT is equivalent to the experimenter’s local time. What is important is only that ZT refers to the environmental time-cycle that an organism is exposed to.
The most common form of environmental cycle that an experimenter will impose is a light-dark cycle. And the most common light-dark cycle that is imposed is 12 hours of light, followed by 12 hours of dark (which then repeats indefinitely). This is often succinctly labelled as “LD12:12” (a Light-Dark cycle, consisting of 12 hours of light followed by 12 hours of dark). This may be equivalently written in some texts as 12L:12D. Most organisms outside of a laboratory setting are entrained to the “LD” (light-dark) rhythms of the natural world. In natural environments, the LD cycle is only 12:12 at the equator and during the autumnal and vernal equinoxes. At other times and locations, the day may be longer than the night (in spring and summer) or shorter than the night (in fall and winter) (see Box 1). Regardless of season or location, however, the total duration of the LD cycle is 24 h. One day’s worth of ZT (e.g., one period of lights on followed by one period of lights off) is denoted with an uppercase Greek letter tau (T). Such a period of zeitgeber time usually lasts 24 hours (unless the scientists are specifically exploring an artificial period like a Martian day) and so, usually T=24h.
To reiterate, the experimental manipulations that chronobiologists impose lead to two new ways of talking about time. When an organism is free-running, we speak of circadian time (CT). When an organism is entrained to an artificially-imposed, environmental daily cycle, we speak of zeitgeber time (ZT).
CT can be additionally described in terms of subjective day and subjective night. A diurnal organism is in the “subjective day” portion of its cycle when it is doing all of the things that it would do during the daytime in a natural setting, such as feeding, being physically active, secreting certain hormones etc. Likewise, a diurnal organism is in “subjective night” portion of its rhythm when it does what it would do during nighttime in a natural setting, such as sleeping or preparing to sleep. It is crucial to note that the situation is precisely reversed for a nocturnal organism. By definition, a nocturnal organism is not active during its subjective day, but rather (as nocturnal) is active during its subjective night. Likewise, a nocturnal organism is passive or quiescent during subjective day. The standard way of thinking about subjective day and night is that they are anchored to an organism’s onset of activity, or the beginning of an organism’s daily schedule of behavior or activity. As such, the onset of activity for nocturnal organism is the start of its “subjective night” (the nocturnal organism aims to wake up and be active at predicted night) whereas the onset of activity for diurnal animals is the beginning of “subjective day” (the diurnal organism aims to wake up and be active at predicted day). By convention, chronobiologists define a nocturnal organism’s onset of activity (the start of subjective night) as CT12. They define a diurnal organism’s onset of activity (the start of subjective day) as CT0. A single cycle of subjective day and night in a given organism takes place over the free-running period (FRP), and the length of one circadian day for an organism is denoted with a lowercase tau (τ).
2.2 Data Collection (Actograms)
One of the standard ways of representing data in circadian biology is in the form of an actogram, a word coined to describe the graphic representation (-gram) of activity patterns (acto-). The term is now widely used to refer to plots of rhythmic data of all varieties, regardless of whether “activity” is measured. Thus, the conventions used in visualizing rhythmic patterns of wheel-running behavior will be virtually identical to those describing, for example, rhythms of hormone secretion, gene expression or protein phosphorylation. A useful introduction to actograms is available in the video “Understanding the actogram.” This video also offers a brief review of the points discussed in the previous section, regarding CT and ZT. For a more detailed and quantitative introduction to CT and ZT, you can also review the video on “Naming Conventions.” Both videos are embedded below. We’ll briefly review the basics in the remainder of this section: revisit these videos after reading this section.
As a first step toward understanding actograms, let’s remind ourselves that a circadian clock is functionally defined as whatever it is that endogenously causes organisms’ activities and processes to exhibit a circadian, temperature compensated, free-running rhythm when zeitgebers are removed. As a result, any easily observable rhythm that an organism exhibits (e.g., regular wake-up time, locomotor activity rhythms, rhythmic changes in body temperature) will only interest us to the extent that it is controlled by the underlying clock. Some of these easily observable rhythms are like the hands of a wall clock. By looking at a wall clock’s hands, we can read the clock’s prediction of the current time, but the gears of the clock – the mechanisms which are genuinely working to keep time, and which cause the movement of the hands – are themselves usually unobserved. Likewise, we can easily observe circadian rhythms in some features of an animal’s behavior: if a mouse is continuously acive it is almost certainly its subjective night whereas if a typical human is sleeping it is probably its subjective night. But the “gears” of the circadian clock itself – the cellular, molecular and genetic mechanisms which are genuinely working to keep time, and which cause the observable rhythm – are not easily observed. (Circadian biologists have indeed developed sophisticated techniques for measuring the gears of clocks, but this took a lot of hard work!)
When humans invented wall clocks, we simultaneously established widespread conventions regarding how to build and read them: 12 o’clock is at the top, 6 o’clock is at the bottom, the long hand counts minutes, the short hand counts hours, etc. Once you know the conventions, you can learn what time a clock is predicting with just a glance at the hands. Circadian biologists haven’t built organisms’ circadian clocks: they have arisen naturally. It is up to us to figure out how to “read” observable rhythms to gain an understanding the underlying clock’s prediction of the time of day. The “hands” of circadian clocks are incredibly diverse: a huge number of observable, organismic processes exhibit circadian rhythmicity and are regulated by the circadian clock. The actograms has been developed as a systematic way of representing a variety of observable rhythms. Once you know the conventions involved in an actogram, you can learn what time an organism’s circadian clock is predicting with just a glance.
When studying animals, circadian biologists commonly treat locomotor activity as a “hand” of the clock, and we’ll use this example to illustrate actograms. In the lab, mice are often given running wheels, which they preferentially use during their subjective night (since mice are nocturnal). We can set up a computer to monitor when the wheel is being turned by the mouse. We can then plot, over time, when wheel revolutions occur. Suppose we put a mouse in an LD:12:12 lighting schedule, and plot a single day’s data on a single horizontal line. It might look roughly like this:
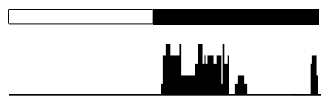
This is not a typical example of a full actogram, but a simplified case to help us introduce some graphical conventions. A lot of information is implicitly contained in this basic graphic. Let’s start with the white and black bars at the top, setting aside the data underneath them. The white and black bars are actually a conventional label used to indicate the light-dark cycle of zeitgeber time (ZT). The white bar represents the period of “lights on,” and the black bar represents the period of “lights off.” The two bars are the same length because our lighting schedule (LD:12:12) involves equal durations of lights on and lights off. So simply by including these bars at the top of the graphic, we’ve indicated that a full day’s worth of data is being plotted, and we’ve given some information about our lighting schedule. Because in zeitgeber time, ZT0 is anchored to the beginning of the light phase, you can read off some key timepoints of ZT from this simple pair of bars, and can make a reasonable estimate of other timepoints:

In Figure 2.2 above, underneath this pair of white and black bars, there is a little bar graph, showing how many times the wheel was spun at each time of day. The higher the black bar, the more times the wheel was spun at that time. Since the mouse is nocturnal, there’s a long period during the day (at left, under the white bar) where no wheel-running is recorded. Instead, all the wheel running occurs during the night (though there are different “bouts” of wheel-running throughout the night).
This simplified graphic, showing one day’s worth of activity, might well be a component of a standard actogram. In a single-plotted actogram, we record from multiple days, and we show successive days on different rows. This actogram shows 4 days’ worth of activity, although many actograms show many more days:

Figure 2.4: A single-plotted actogram showing 4 days of activity.
Things are not much different in a double-plotted actogram: this is another format that circadian biologists often use. We still show successive days beneath each other, on different rows in the same column. However, we also show two successive days next to each other, in different columns on a single row. This results in a duplication of data, as shown below:

This graphic gives an indication of how double-plotted actograms are composed. But this duplication of data might look like a waste of ink: the case shown above does not provide a very good illustration of why circadian biologists have found the double-plotted format useful. In fact, double-plotted actograms are most often used (and most useful) when we are plotting data from an un-entrained (free-running) organism. We will discuss an example shortly. For now, notice that the examples shown so far show entrained activity, when a zeitgeber is present (as indicated by the white and black bars). Because the organism is entrained, these actograms do not provide us with any information about its free-running period (FRP). But we can use the same style of graphic to show data about organisms’ activity when they are free-running in the absence of a zeitgeber, in constant conditions.
Let’s suppose we put an organism into 24 hours of constant darkness for about 20 days. To keep things simple, we will eliminate the fine details of the precise amount of locomotor activity recorded at each timepoint. Instead we will just use solid black lines to represent the whole active phase. Two different, schematic examples of what we might find are shown below. Both employ a single-plotted actogram:

In both actograms, the full black bar at the top indicates that the organism is in constant darkness and is thus free-running. In the actogram on the left, you’ll notice that the black marks, representing activity, drift slightly to the right as you progress from the top (beginning of the recording) to the bottom (through successive days of the experiment). This indicates that the organism is reliably becoming active slightly later each day. From this pattern we can instantly infer that the organism’s FRP is longer than 24 hours. Circadian biologists would say that this organism has a long free-running period, (or simply, a long period) since it is greater than 24 hours. And because the FRP is greater than 24 hours, but the actogram shows only 24 hours of data on each row, as enough days pass the activity pattern wraps around the edge of the graphic and begins appearing on the left side. In the actogram on the right, you’ll notice that the black marks drift to the left in a regular way. This indicates that the organism is reliably becoming active slightly earlier each day and thus the organism’s FRP is shorter than 24 hours. Circadian biologists would say that this organism has a short free-running period (or simply, a short period). And because the FRP is less than 24 hours, but the actogram shows 24 hours of data, the activity pattern eventually wraps around the edge of the graphic.
These single-plotted actograms help provide an illustration of why double-plotted actograms have become popular formats. It is somewhat difficult to read these actograms, due to the fact that the activity wraps around the edge of the graphic. This is very likely to occur when we plot data from a free-running organism, since organisms rarely have an FRP of exactly 24 hours. And so the double-plotted actogram duplicates data simply for the sake of making it convenient to see a whole day’s bout of activity in one glance. Here are double-plotted versions of the actograms above:
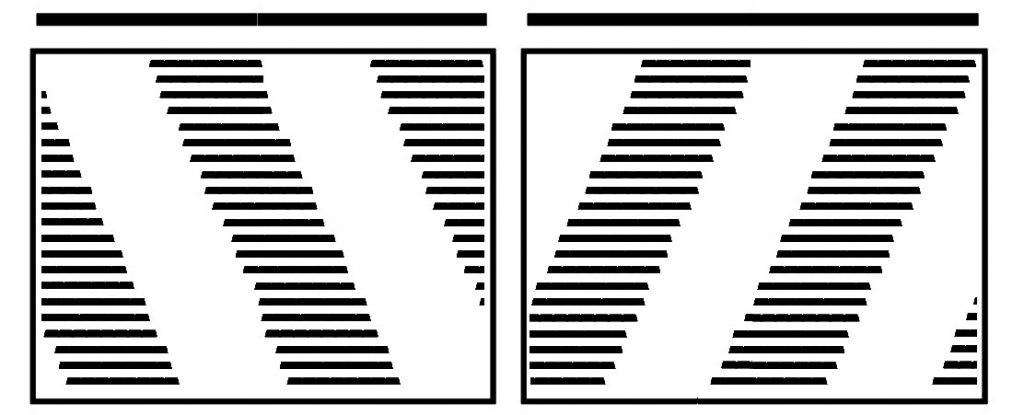
Remember: when an organism is in constant conditions, we cannot speak of zeitgeber time (ZT), since there are no zeitgebers present. Instead, we speak of circadian time (CT). Each cycle of circadian time consists of, and can be divided into 24 circadian hours, even if the organism’s FRP is more or less than 24 hours of actual solar time. And remember, CT is typically anchored to onset of activity: for a nocturnal organism, the onset of activity is defined as CT12, and for a diurnal organism, the onset of activity is defined as CT0. So if you know in advance whether an organism is nocturnal or diurnal, then a glance at the actogram for a free-running organism can fix a few time-points in CT, and lets you make some reasonable estimates about other timepoints. This is illustrated below, using a double-plotted actogram from a free-running, nocturnal organism. Notice that we have returned to showing the fine detail in the pattern of running. Consider also that our labels would be different if these activity data were known to come from a diurnal organism.

In this brief set of examples, we’ve supposed that the black marks represent locomotor activity: we read these activities as the “hands” of the circadian clock. But many observable rhythms could serve as the “hands” of the clock, and most of them can be represented using the basic format of the actogram. We might use the bar graphs or black marks to record when cell division is occurring in some colony of bacteria, or to when body temperature is above the daily average, or when hormones like melatonin are being secreted, etc. While this will change the fine details of the actogram, it will not change the essential format of the actograms shown here. This provides an illustration of how we can use actograms to depict what the hands of the clock are doing. Once data are put into the basic actogram format, a glance at the actogram can tell you whether the organism is entrained, or whether it is free-running – and if so, whether it has a short or a long FRP. This isn’t quite as precise as learning to read a wall clock: a glance at the actogram cannot tell you whether the FRP is precisely 24.8 hours, for example. To know that, you have to run some calculations on the raw data. But actograms easily convey crucial information about entrainment and FRPs, and as a result, they have become a canonical form of data visualization in circadian biology.
The foregoing has been an overview of how actograms offer one standardized way of reading the “hands” of a circadian clock, and how an actogram encodes information about ZT and entrainment, and about CT and free-running rhythmicity. With this in place, we can hint at an important phenomenon – and a complication – called masking. Masking occurs when something in the environment directly affects activity (or whatever hand of the clock we are plotting in the actogram). For example, if an investigator changes the bedding of a mouse in its cage during the day, the mouse may start running in its wheel. This induced activity (e.g., positive masking), however, does not mean that the mouse’s clock thinks that it is night. Conversely, if there is loud noise at night, a mouse might be inhibited from running (e.g., negative masking). This lack of activity is not an indication that the clock is in subjective day. Thus, masking raises the possibility that what we see in the actogram is not a reflection of the underlying clock mechanism but is partly or wholly environmentally-induced. For example, an observed 24 h rhythm may be entrained, but it might also be a spurious or epiphenomenal rhythm in response to uncontrolled environmental factors. To continue our analogy: masking occurs when (i) the observable phenomena which we have selected to read as “hands” of the clock have become uncoupled from or are no longer faithfully tracking the gears, even though (ii) they exhibit a clearly circadian rhythmicity. Put another way, masking occurs when an organism merely appears to be entrained to a zeitgeber when, instead, the state of its circadian clock has not been altered. In such cases, an actogram might be misleading. We will discuss masking in more detail in §3.2 below. For now, it suffices to say that if we want to get a good reading of the circadian clock, we need to rule out the possibility of masking.
Click to copy citation: Kao, K., Loi, J., Sanden, H., Kim, J., Lee, J., Nudell, V., Wu, L., Golden, S.S., Gorman, M., & Sheredos, B. (2016, September 22). Introduction to chronobiology. Retrieved from https://bioclock.ucsd.edu/portfolio-item/an-introduction-to-chronobiology/.